In recent years, due to climate change, environmental sustainability programs have been created that require the search for alternatives to reduce air emissions and reduce fuel consumption. The combustion industry is looking for an effective solution to increase the life cycle of its equipment and improve combustion efficiency without neglecting safety or savings. The efficiency of the medium pressure natural gas burners associated with chemical energy, which is converted into heat, that is, the hotter the gaseous products of combustion, the more efficient the burner. The work analyzes the combustion process and their chemical reactions; combustion products obtained in accordance with various conditions; combustion characteristics and factors that influence; and measurement methods needed to optimize combustion and their equipment. In the metallurgical production of nickel, optimization of the burners is very important due to the high temperature used in the reverberatory furnace. That is, the use of a large excess of air would be unfavorably energetic, and the use of a very low excess would result in small intimate mixtures of air and fuel, followed by the release of unreacted reagents and the formation of toxic gases arising at high temperatures. For this reason, the results of this work are an important source of information. The innovation is to automate the use of non-invasive sensors to control the combustion process. The sensors play a fundamental role in the industry, because the information they provide supports the enhanced use of data in the enterprise and increases productivity, efficiency, and safety.
1 Introduction
Combustion is a chemical process in which oxidation of the fuel components occurs, in our case, natural gas combined with oxygen (O2) from the air. If natural gas contains high sulfur content when burned, sulfur oxides are formed, which in turn form corrosive products; therefore, if combustion water is allowed to condense, sulfur oxides dissolve in it. On the other hand, if natural gas contains nitrogen and this is combined with oxygen, it forms nitrogen oxides (NOx) [1].
The fuel is given two combustion heat values: The higher calorific value: In this, the water from the combustion products is extracted in a liquid form. In the lower calorific value, the water from the combustion products is extracted in the form of steam.
All this in view of the latent heat of vaporization is not useful in combustion processes [2].
To achieve efficient combustion, sufficient combustion air is needed but not excessive; meaning that a mixture should be produced within the flammability range. In the case of natural gas, the range goes from 64 percent to 247 percent. The combustion air must fulfill its function under the slogan of the three Ts: time, in a short period; temperature, at high temperatures; and turbulence — you must create a very turbulent flame [3].
Turbulence is an important aspect to consider in a nickel reverberatory furnace as the working temperature exceeds 1,450°C. Natural gas and well mixed air will cause complete combustion; therefore, the flame temperature will be high according to the desired atmosphere for the furnace, and the burning time will be short. Medium pressure natural gas burners play a very important role in this research since, after the study, an automation of them will help us to obtain a complete combustion [4].
Analyzing the composition of combustion gases based on the amount of air with possible variables leads us to conclude the importance of adhering the right amount of air. See Figure 1.

With an excess of air (excess oxygen), excess gases (N2 and O2) causes a decrease in the outlet temperature and a low yield that has been represented in Equation 1:
With a lack of air (lack of oxygen), natural gas does not burn completely, and they appear unburned in the flue gases, as shown in Equation 2:
The ideal stoichiometric chemical reaction with the necessary oxygen is described in Equation 3:
The composition of the flue gases, depending on the excess/lack of oxygen, is shown in Figure 2.
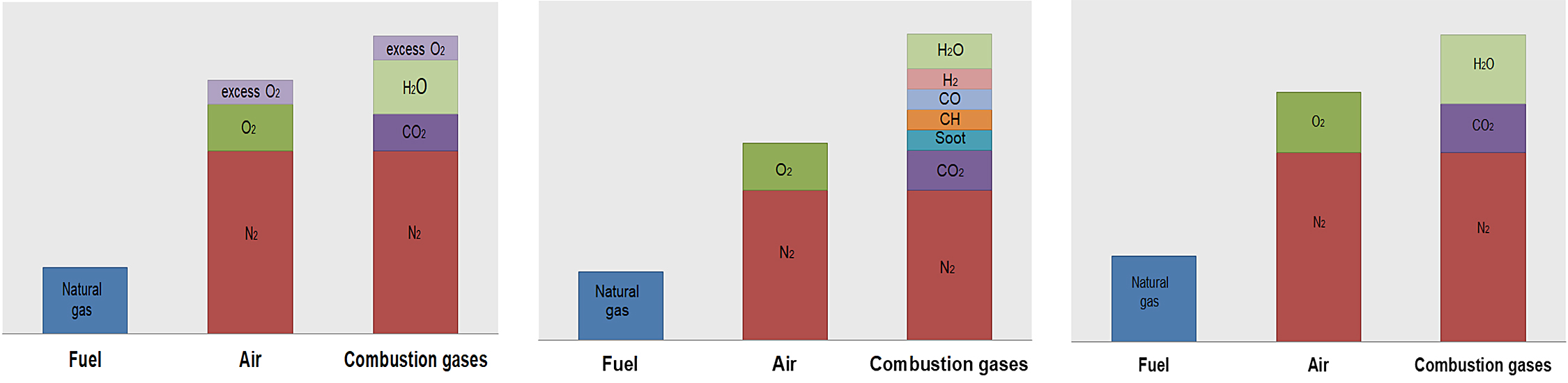
Nitrogen oxides (NOx) are also produced at high temperatures (>1,450°C). The O2 and N2 molecules dissociate, and free atoms are formed that react with each other, as shown in Equation 4:
The air used for combustion is a mixture of oxygen and nitrogen. To supply 1 kg of oxygen for combustion, it is necessary to provide 1/0.2315 = 4.32 kg of air. In this quantity, there will be 4.32 kg · 0.7685 = 3.32 kg of nitrogen, which does not directly interfere with the combustion process, but this is always present.
The medium pressure burners do not do a good job in the mixture between natural gas and air, although we achieve the best turbulence conditions. For this reason, it is necessary to create an algorithm based on the thermal function of the reverberatory furnace.
To ensure complete combustion, it is necessary to supply more theoretical air. This ensures any molecule of natural gas can find the necessary oxygen molecules for combustion. Total air would be equal to theoretical air plus excess air. Figure 3 describes the composition of the gas at the outlet as a function of the air flow for natural gas [5].

2 Materials and methods
The literature tells us the efficiency of reverberatory furnaces is defined as the relationship between the heat transferred to the required process fluid and the energy content of natural gas. The efficiency may vary depending on the individual furnace design, the furnace load, the excess air, the flue gas temperature, and the furnace maintenance. A loss of efficiency of 1% can be caused by a 2% increase in excess oxygen or a 23°C increase in the temperature of flue gases [6].
The general efficiency of the furnace consists of two cases: the combustion efficiency — which is part of the total energy available in the combustion chamber after the combustion process, and the efficiency of the furnace — which depends on the design and its operation.
To make any of the two cases effective, we need to optimize resources. If we optimize manually, the manual adjustment of the furnace will determine the point of minimum losses and will change the working conditions at this time. If we optimize automatically, the control system will work by continuously determining the point of minimum losses at a given load and changing the working conditions at this time [7].
During automation, we must take into account the temperature of the flue gases. The amount of energy lost through the chimney depends on the amount of excess air and the temperature of the outlet gases. The exit temperature is a consequence of the load, the infiltration of air, and the state of the heat transfer surfaces.
The literature indicates that, to start and optimize a reverberatory furnace, a table must be created where we present the temperature of the gas at the outlet depending on the load. This serves as the basis for assessing the subsequent characteristics, if the temperature rises above this baseline; this indicates a loss of efficiency. With each temperature rise of 23°C, the efficiency of the reverberatory furnace decreases by 1%.
The contamination of the heat-transfer surfaces in the air heater, the progressive oxide coating inside the furnace tubes, and the progressive soot coating (see Figure 4) on the outside of the reverberatory furnace pipe are the reasons why the outlet temperature can increase [8].

For this study, we worked with the ex situ method. The installation is shown in Figure 5.
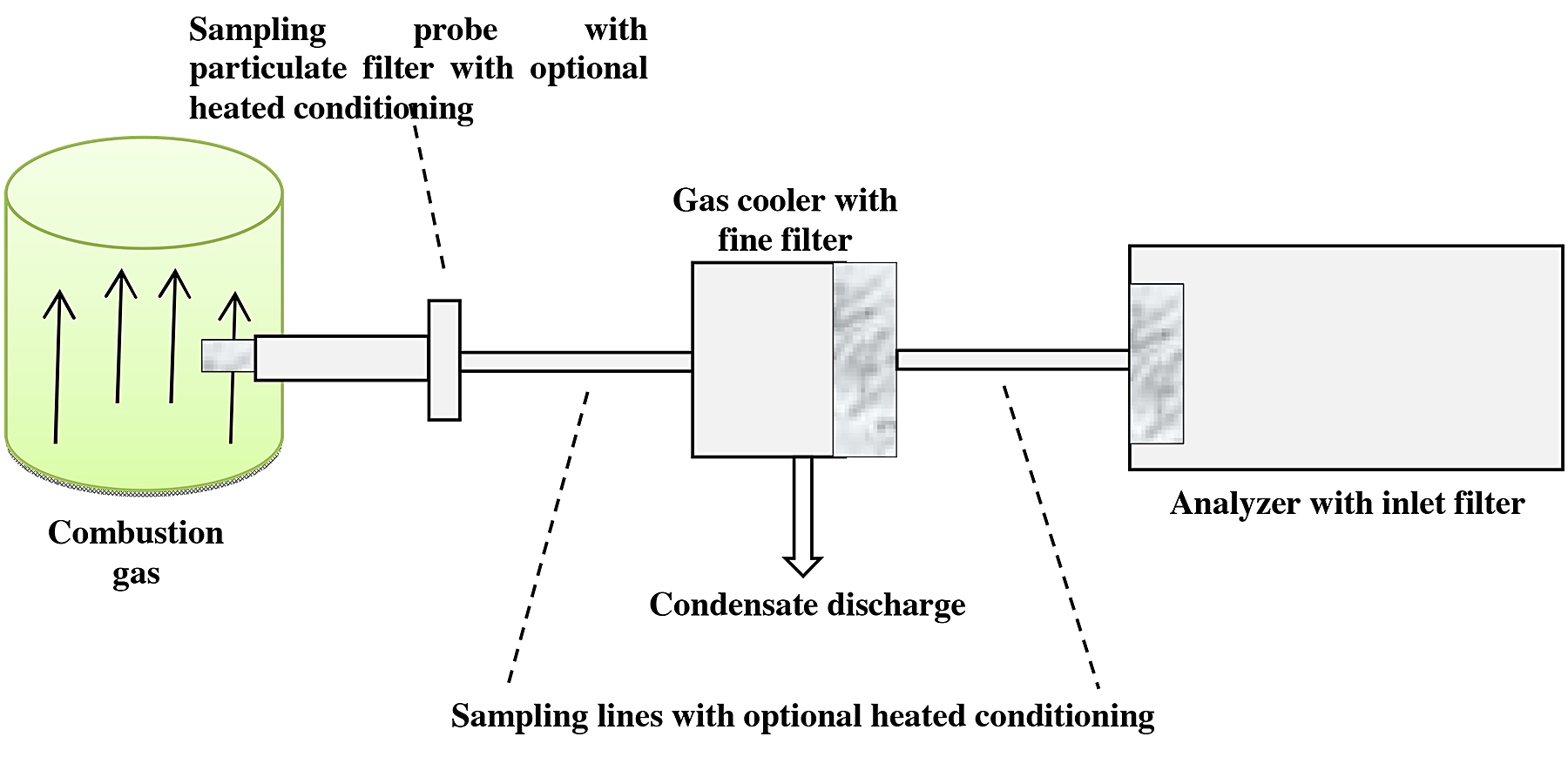
A representative sample of the gas to be analyzed (combustion gas) was extracted. Firstly, this gas was passed through a conditioning unit that consists of cleaning the particle gas, and it was then cooled to a fixed temperature below the dew point to dry it. Finally, the gas passed to the analyzer (see Figure 5).
The combustion gas is extracted by means of sampling probes introduced inside the duct and a pump that aspirates the gas to be analyzed. The maximum analysis temperature was 1,500°C and was measured with sensors that also helped measure the pressure [9].
The unit consists of an analyzer that is separated and protected; the conditioned gas has a defined state thus comparable.
The following measurements were made for the analysis of flue gases: % of oxygen (O2) as an individual control indicator, carbon monoxide (CO) as an individual control index, and the measurement based on a combination of % oxygen (O2) and ppm of carbon monoxide (CO). Table 1 shows the results measured on a dry basis.

3 Results and Discussion
The analysis of oxygen as an index of individual control in any combustion process gives us the idea there is a balance between fuel, carbon monoxide, and oxygen, as we see in Equation 5:
The oxygen shifts the equilibrium to the right. In a well-mixed system with excess air, the concentration of unburned fuels must be less than the concentration of carbon monoxide. If CO is controlled within satisfactory limits, the hydrocarbon concentration will necessarily be low.
In the same way, it is very important to control the content of oxygen in combustion gases since this implies an implicit control of carbon monoxide in equilibrium with oxygen. However, the balance depends largely on the air-fuel mixture. An air deficiency at a point in the combustion zone can result in an increase in unburned fuels and CO, an inefficient atomization due to fouling in the burner can have a similar effect.
This means a control over oxygen does not necessarily ensure a control over the emissions of CO and hydrocarbons (even when the excess of O2 with the load is well characterized) [10].
The CO as an individual control index is based on maintaining a constant flow of CO at all charges. This function is determined based on an analysis of the composition of natural gas, excess air, the temperature of combustion gases, tests on the reverberatory furnace to determine the shape of the ppm curve of CO vs. excess air (see Figure 6). The ranges of CO range from 0 to 1,000 ppm. If we receive signals of a change to 100 ppm of CO, this change is accompanied by a change in the % of O2 of 0.1, and this change in turn is equivalent to a % of 0.5 of excess air [11].
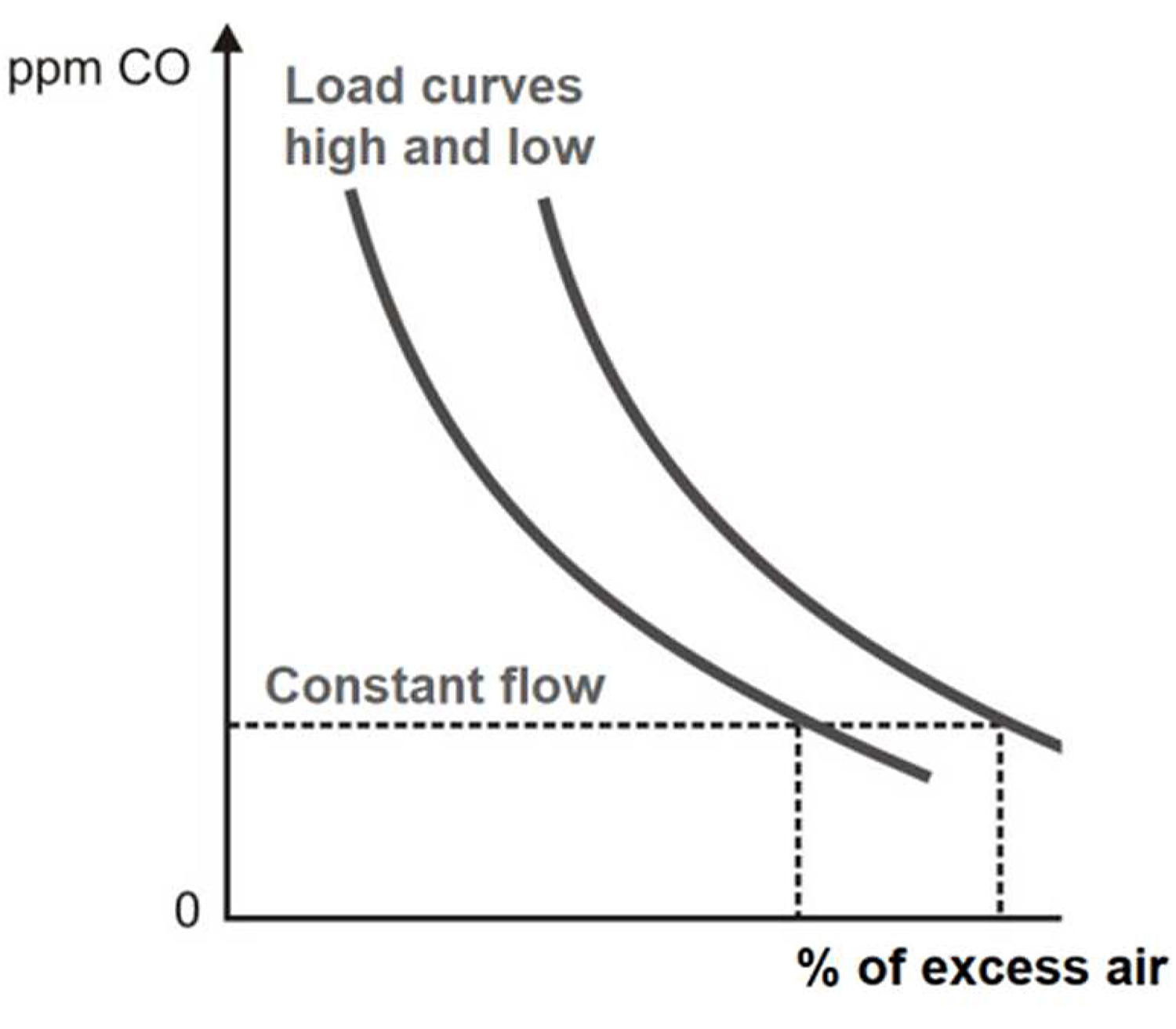
Control based on combination of % O2 and ppm CO. Control by CO only demonstrates certain shortcomings such as the formation of CO, which can be affected by rapid changes in fire velocity or excess air levels.
Poor burner operation may cause the following sequence: The CO increases; more combustion air is added, but the burner is still in poor condition. This greater air causes a dilution of CO; the concentration of CO returns to the flow.
It is working with the correct concentration of CO, but the excess air is too large, and it is not possible to see it. To detect it, you must know the % O2 in the flue gases.
A control system cannot improve the basic performance of a reverberatory furnace or its burners.
A good control system can bring the operation of the furnace close to its best operating level for a particular load and other environmental conditions. At a lower load, greater % of O2 is required to burn all the fuel (see Figure 7) [12].
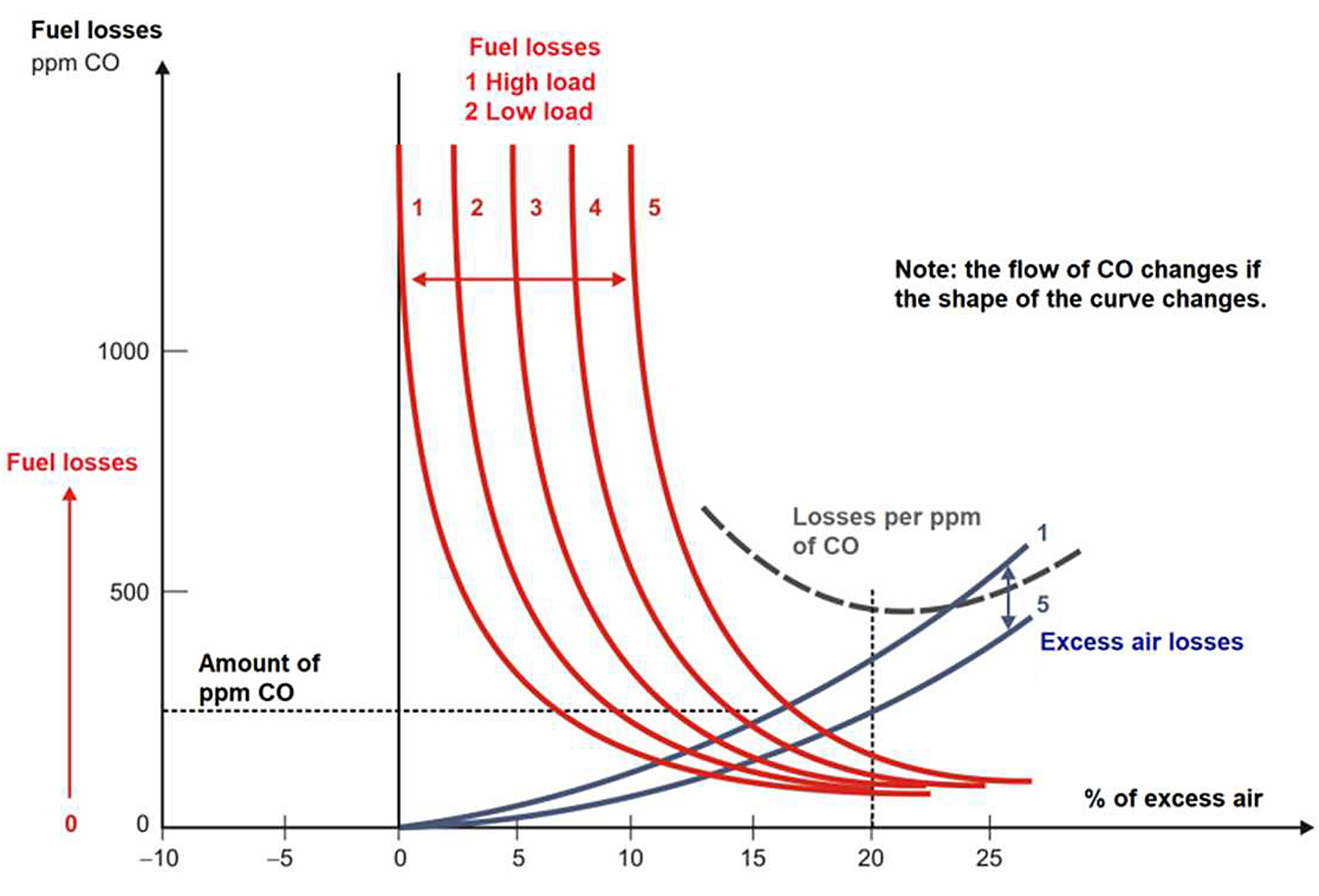
With the results of the analysis of combustion gases, we determine that we can control the % of O2 and the amount of ppm of the fuel described:
The control of O2 is obtained by setting a certain percentage of excess air from the measurement of % O2. Under test conditions, it is established that the excess O2 flow must be so that the losses in the reverberatory furnace are minimal. A programmed O2 flow should be used to be set based basically on the furnace’s fire demand. A small safety margin (3 to 4% excess air) is added to cope with variations in a reverberatory furnace or burner operation regarding the test conditions.
Control from the ppm of fuel in the flue gases: We could assume that, for furnace loads and for better economic performance, we should keep this measure at a relatively constant value since the excess air needed would automatically increase for different loads. But from data obtained, it is observed that the temperature in the flue gases increases at a higher load in the furnace, and a change in the shape of the fuel loss curve vs. excess air is observed when the load changes. As a recommendation, the optimum ppm content for each load value should be determined from tests carried out in the reverberatory furnace. A CO flow programmed with the load would be used, as is done with O2 (see Figure 8) [13,14].

4 Conclusion
The melting temperature is the most important factor that affects the efficiency of the main technological operations in gas reflecting furnaces. The temperature determines the quality of the future product, the effectiveness of the multi-stage refining processes in a liquid metal bath during the fusion process, as well as the intensity of the saturation of the fusion gas, its obstruction with impurities, and irreparable losses [15].
The burners are an essential part of a furnace, and it is necessary to optimize them based on combustion processes to find the ideal balance when mixing the fuel with the air, to ensure complete combustion, and therefore a desired temperature in the required time.
One of the necessary requirements is the supply of a larger amount of theoretical air so any fuel molecule can find the oxygen molecules necessary for combustion. This excess of air and oxygen depends on the fuel. In the case of natural gas, the minimum % of excess air must be between 4 and 16 percent, and the % of O2 present in the combustion gases is 0.5 to 3.2 percent. This excess air will come out in the flue gases. The percentage of oxygen (% O2) in the flue gas can be used to determine the percentage of excess air, and we can use the % carbon dioxide (% CO2) to determine the percentage of excess air. The amount of excess air required will depend on the type of fuel, the burner design, the characteristics and preparation of the fuel, the reverberatory furnace design, the load as a percentage of the maximum load, the degree of air penetration, and the environmental conditions.
References
- Warnatz J, Maas U, Dibble R 2006 Combustion 4th edn. (Heidelberg: Springer).
- Fetisov VG, Nikolaev A K, Lykov UV 2017 Experimental studies for determining gas flow rate accidental release on the linear part of the pipeline IOP: Earth and Environmental Science 87 11-1.
- Aslanyan GS, Maikov I L 1998 Numerical simulation of turbulent gaseous combustion in axially symmetric combustion chambers Combust Explos Shock Waves 34 369–377.
- Kim H, Lee SY, Kim H J. et al. 2020 Numerical study on the effects of tumble and swirl on combustion and emission characteristics of an LPG direct injection engine International Journal of Automotive Technology 21(3) 623–632.
- Lisienko V G, Malikov YK, Surganov KA et al. 2008 Design and automation of a modern reverberatory heat-treatment furnace Metallurgist 52 714–718.
- Quiroz Cabascango VE, Bazhin V Yu 2020 Nickel oxide reduction in CO/CO2 gas mixtures in reverberatory furnaces Journal of Physics: Conference Series 1515 022028.
- Mamalis S 2019 Low-temperature natural gas combustion engines In: Srinivasan K, Agarwal A, Krishnan S, Mulone V (eds) Natural Gas Engines. Energy, Environment, and Sustainability (Singapore: Springer).
- Lissianski V, Zamansky V, Gardiner W 2000 Gas-Phase Combustion Chemistry (New York, Springer).
- Thornton JD, Chorpening BT, Sidwell T G, et al. 2009 Flashback detection sensor for hydrogen augmented natural gas combustion ASME Turbo Expo: Power for Land, Sea, andAir 739–746.
- Ibadullaev TB, Arutyunov VA, Levitskii I A et al. 2007 Simulation of natural-gas combustion in a blast-furnace tuyere Steel in Translation 37 924–928.
- Solonenko VV, Protopopov EV, Feiler S V et al. 2017 Oxidation of molten impurities in converters by means of combustion flames: Thermodynamic principles. 1. Thermodynamic analysis of processes in natural-gas combustion Steel in Translation 47 449–455.
- Bains P, Psarras P, Wilcox J 2017 CO2 capture from the industry sector Progress in Energy and Combustion Science 63 146–172.
- Fetisov VG, Nikolaev AK, Lykov YV 2018 Aggregative simulation method for implementing mathematical models for gas transmission systems IOP Conference Series: Materials Science and Engineering 327(2) 022033.
- Galnbek AA, Shalygin LM, Shmonin Yu V 1990 Calculations of pyrometallurgical processes and non-ferrous metallurgy equipment (Chelyabinsk: Metallurgizdat).
- Cheremisina OV, El’-Salim S Z 2017 Modern methods of analytical control of industrial gases Journal of Mining Institute 228 726-730.
Published under license by IOP Publishing Ltd. Journal of Physics: Conference Series, Volume 1728, International Conference on Complex equipment and quality control laboratories (CEQCL) 2020 14-17 April 2020, Saint Petersburg, Russian Federation. (https://iopscience.iop.org/article/10.1088/1742-6596/1728/1/012019) This is an open access article under under the terms of the Creative Commons Attribution 3.0 license (https://creativecommons.org/licenses/by/3.0). It has been edited to conform to the style of Thermal Processing magazine.