The paper concerns the effect of annealing time and temperature on the properties of the nitride layer on X37CrMoV51 tool steel used in the extrusion aluminum industry. Samples made from X37CrMoV51 steel were hardened and tempered, and then nitrided at 530°C. After nitriding, the samples were annealed in a furnace at 470°C for 8 hours, 12 hours, 24 hours, 30 hours, and 60 hours, and additionally for 20 hours at 270°C. The samples were tested for structure, hardness, and abrasion immediately after nitriding and again after annealing. It was found that annealing the nitrided samples leads to degradation of the nitride layer, accounting for the decrease of hardness. The annealing of the samples at 470°C for more than 12 hours causes a decrease in mean hardness value from 1,176 HV to 1,103 HV, and annealing the samples more than 30 hours at this temperature leads to a decrease in hardness to 964 HV. The changes in nitrogen content in the white (compound) and diffusion layers and the resulting consequences of changes in phase composition and properties were evaluated. Annealing more than 30 hours at 470°C caused the white layer to disappear and the average nitrogen content in the diffusion layer to decrease to the level of about 5–6 at%.
1 Introduction
Nitriding of tool surfaces is one of the most commonly used types of heat treatment of tool steels. The nitriding process increases the hardness of the surface layers as well as resistance to abrasion and corrosion [1–4].
The properties achieved by nitriding may change due to the use of the dies and their annealing. During the extrusion process, the die is exposed to high temperatures that affect the surface condition. Before the dies are placed in the press, they are heated, which also affects the condition of the nitride layer.
Dies for extrusion of aluminum alloys are subjected to repeated regeneration by secondary nitriding, which results in multiple high-temperature treatments. The need for regeneration results from the abrasion of the nitrided layer from the surface of the calibrating bearing of the dies during their exploitation.
Nitriding consists in diffusing nitrogen into the surface layer of steel. It is carried out in the temperature range 500–650°C in an ammonia atmosphere. Above 400°C, the ammonia decomposes according to the NH3 → 3H + N reaction. Atomic nitrogen diffuses into the steel and forms nitride phases. At the temperature of 591°C, the nitride layer consists of three phases: ε-Fe2N nitride, γ′-Fe4N nitride, and nitrogen ferrite, which contains 0.01% nitrogen at room temperature. At the temperature of 600–650°C, the γ phase is formed, which, when slowly cooled down to the temperature of 591°C, transforms into eutectoid ε + γ′ [5–9].
The hardness of the nitride layer reaches the level of 1,100–1,200 HV and should maintain this level when reheated to working temperatures of 500–600°C. The nitride layer of tempered steel is wear-resistant.
The nitriding process, which lasts from about 20 to 50 hours, produces a layer with a thickness of 0.2–0.4 mm. Increasing the nitriding temperature causes an increase in the thickness of the nitride layer; however, it reduces its hardness, which adversely affects the resistance to abrasion. Literature data indicate that lower nitriding temperature favors high hardness of the nitride layer and good resistance against erosion and corrosion resistance [1–3].
The effect of nitrogen presence in the atomic structure of steel is the formation of nitrides and the interstitial location of nitrogen in the crystal lattice. Depending on the concentration of nitrogen, different stoichiometric nitride compositions can be found. Nitrides as compounds, such as titanium nitride [10] or boron nitride [11], are characterized by high hardness and refractory properties and are used in the production of cutting tool blades, refractory laboratory vessels, and protective coatings. Gallium nitride was used in the production of blue laser [12]. Like some oxides, nitrides can absorb hydrogen and are considered for hydrogen storage, e.g., lithium nitride [13].
Depending on the concentration of nitrogen, there are different stoichiometric nitride compositions in steels. Apart from nitrides formed as a result of the connection of nitrogen and iron, such as Fe2N, and Fe4N, CrN nitrides are also formed in steels containing chromium [14–19]. The formation of a CrN phase results in the reduction in the corrosion resistance of the nitride layer in chromium-containing steels. The CrN phase is formed during nitriding above 500°C and occurs frequently when using traditional methods of nitriding [1,2]. CrN precipitations, dissolved in ferrite, are very hard. The temperature increase causes them to undergo coalescence, which leads to the loss of coherence with the lattice and the decrease in hardness, as well as initiate changes in the profile of long-range internal stresses. These changes already take place during nitriding.
Depending on the nitrogen content, iron nitride phases with different structure and properties can be formed. Iron has five nitrides observed at ambient conditions: Fe2N, Fe3N4, Fe4N, Fe7N3, and Fe16N2 [15,19,20]. They are crystalline, metallic solids. Lately, it was put forward that the phase diagram of iron nitrides can be extended further to even more N-rich compounds, such as the γ″-FeN and the γ″′-FeN phases [21].
All iron nitrides are metallic conductors, and they are metastable with respect to decomposition into Fe and N2. The decomposition is limited by kinetic barriers. Atomic nitrogen can be dissolved in the body-centered cubic (bcc) lattice of α-Fe to a concentration of about 0.4 at% N without much distortion of the lattice. When more than 2.4 at% N is dissolved in pure Fe, the bcc lattice undergoes a tetragonal deformation. In the composition range up to about 11 at% N, the iron nitride compound is called nitrogen martensite α′. This phase has a body-centered tetragonal (bct) structure with lattice parameters depending on the nitrogen content. The N atoms occupy randomly octahedral hollow sites in the Fe sublattice. At saturation, nitrogen martensite has the Fe8N composition. The α′-Fe8N can transform into the α″-Fe16N2 phase. In this phase, the N atoms are ordered. It can be formed under special conditions from Fe, however, not in its pure form. The α″-Fe16N2 phase attracted considerable attention because of a possible very high saturation magnetization, reported to vary between 2.4 T and 3.2 T [16]. The next phase of the nitrogen content is the γ′-Fe4N phase (roaldite), which is cubic, with the Fe sublattice arranged in a face-centered cubic (fcc) structure and nitrogen atoms occupying the body-centered position 1/4. As indicated in the phase diagram, this phase has a narrow composition range around 20 at% N. The lattice parameter is 3.795 Å, and the saturation magnetization was reported to be between 1.8 T and 1.9 T [17]. A saturation magnetization value in this range is slightly lower than the one of pure iron (2.21 T), making this phase somewhat less attractive in comparison with Fe.
The authors of the paper [21] point to the important role of carbon in the process of phase changes in the nitride layer and its influence on the internal stress in this zone. Carbon diffusion implies a complex precipitation sequence and a thermodynamic evolution that modify the volume change during nitriding. The transformation of initial carbides into nitrides decreases the kinetics of nitriding and is counteracted by the precipitation of cementite. Surface decarburization involves a decrease in the volume fraction of cementite during nitriding, leading to an unloading of the surface and thus reducing residual stresses. During nitriding, a surface was subject to mechanical loading-unloading through volume changes. The distribution of residual stresses is mainly governed by the thermochemical modifications due to nitrogen and carbon diffusion.
Studies show the abrasion resistance of the nitride layer depends on the type of nitriding method [22]. Studies by G. Kugler et al. [23] have shown the presence of a compound (white) layer protects the surface of dies against chemical reaction with hot aluminum [24]. It was found that annealing of the ε-Fe3N phase leads to the precipitation of the γ′-Fe4N phase. In this paper, it was also found that long annealing of ε phase causes its homogenization.
In general, it should be emphasized that any time a surface-nitrided tool is exposed to high temperatures, it leads to changes in the nitride layer. In combination with the changes that occur in the substrate, consisting in lowering the density of defects (recovery, polygonization) and the precipitation or decomposition of carbides, as well as their coagulation, this affects the properties of the nitride layer. In particular, the hardness and abrasion resistance of the nitrided surface change. Reducing these properties reduces the life of the dies and increases production costs.
The scope and scale of changes affecting the durability of the dies is of crucial importance due to the practice of using the dies in industry. From this point of view, the more stable the hardness of the nitrided layer is, the better the prognosis of the durability and utility of the die.
Continuous development of the aluminum market causes the demand for dies to increase. According to the prediction of the International Energy Agency, between 2023 and 2030, the demand for aluminum will increase by more than 50 percent, due to, among other things, the rapid development of LED lighting and the development of the automotive industry [25]. The largest aluminum products’ production plant in Poland is Grupa Kęty S.A. S.A., which is a company with a long tradition [11]. Grupa Kęty S.A. assumes that, by 2019, it will become the leader in the production of aluminum profiles in Poland with sales of 1,364 million PLN in the Polish aluminum market [26].
The development of the aluminum industry generates the development of production and heat treatment of dies necessary for the production of aluminum profiles. Due to the importance of the die durability issue, the nitriding process is constantly being improved by companies producing nitriding furnaces. Therefore, all research on this subject contributes to the broadening of the knowledge on this subject and to the improvement of the nitriding technology.
In this paper, the influence of temperature on the properties of the nitride layer of tool steel X37CrMoV51 (1.2344 tool steel) was analyzed. Annealing was carried out for different time periods (8-60 hours), and the influence of the given heat-treatment conditions on the structural effects and properties of the nitride layer was evaluated.
2 Materials and Methods
The tested samples were nitrided in a Nitromax furnace under industrial conditions.
Samples made from X37CrMoV51 steel were hardened and tempered and then nitrided at 530°C, according to Grupa Kęty S.A.’s (Kęty, Poland) proprietary technology based on the NITREG technology, under conditions in which industrial dies in Grupa Kęty S.A. are nitrided. During primary nitriding, a 120 to 140 µm thick diffusion layer should be formed on the dies. The thickness of the white layer should be between 4 and 6 µm, and the hardness should be higher than 1,000 units.
After nitriding, the samples were annealed in a furnace at 470°C for 8 hours, 12 hours, 24 hours, 30 hours, and 60 hours, and additionally for 20 hours at 270°C. The samples were tested according to the following procedures for structure, hardness, and abrasion resistance immediately after nitriding and again after annealing.
The research was carried out on cross sections. After cutting out the sample, it was sanded with grade #300 and #1200 abrasive papers and polished with diamond paste with grain sizes of 9 µm, 3 µm, and 1 µm. The final polishing was carried out using the silica suspension OP-S according to Struers company procedures. In the next step, the samples were etched in Nital reagent with the following composition: 5 ml HNO3 + 100 ml C2H5OH.
The microstructure of the samples was examined using the Olympus GX-51 optical microscope (Cracow, Poland) and the Hitachi SU-70 scanning electron microscope (Cracow, Poland) equipped with Energy Dispersive X-Ray Analysis (EDX).
The microhardness of the nitride layer was examined using the Shimadz HMV-G apparatus (Cracow, Poland) by means of Vickers’ method with the load of 0.9807 N.
Chemical analyses in the microarea of nitrided steel and iron oxides formed as a result of gas nitriding were carried out using an electron probe microanalyzer Jeol SuperProbe JXA-8230 (Cracow, Poland) equipped with Wavelength Dispersive X-ray analysis (WDS). The tests were performed in the Critical Elements Laboratory of AGH -KGHM at the Faculty of Geology, Geophysics and Environmental Protection of the AGH University of Science and Technology in Krakow. Investigations of nitrided steel were carried out with the use of an electron probe microanalyzer with the acceleration voltage of 15 kV and the amperage of 50 nA, whereas, during the measurements of iron oxides, the amperage was 40 nA. The time of analysis of each element lasted 20 seconds in peak maximum position and 10 seconds in background position before and after the peak. The beam size was <1 µm. The following lines and standards were used for steel analysis: BN (NKα), Fe (FeKα), Mo (MoLα), V (VKα), Cr (CrKα). The following lines and standards were used for the analysis of iron oxides: BN (NKα), fayalite (FeKα), Mo (MoLα), V (VKα), Cr2O3 (CrKα).
3 Results
Studies on the structure of samples directly after nitriding showed that a white (compound) layer was formed on their surface (Figure 1). The thickness of this layer was estimated at about 7-8 µm, while the depth of the diffusion layer was about 70 µm (Figure 1).
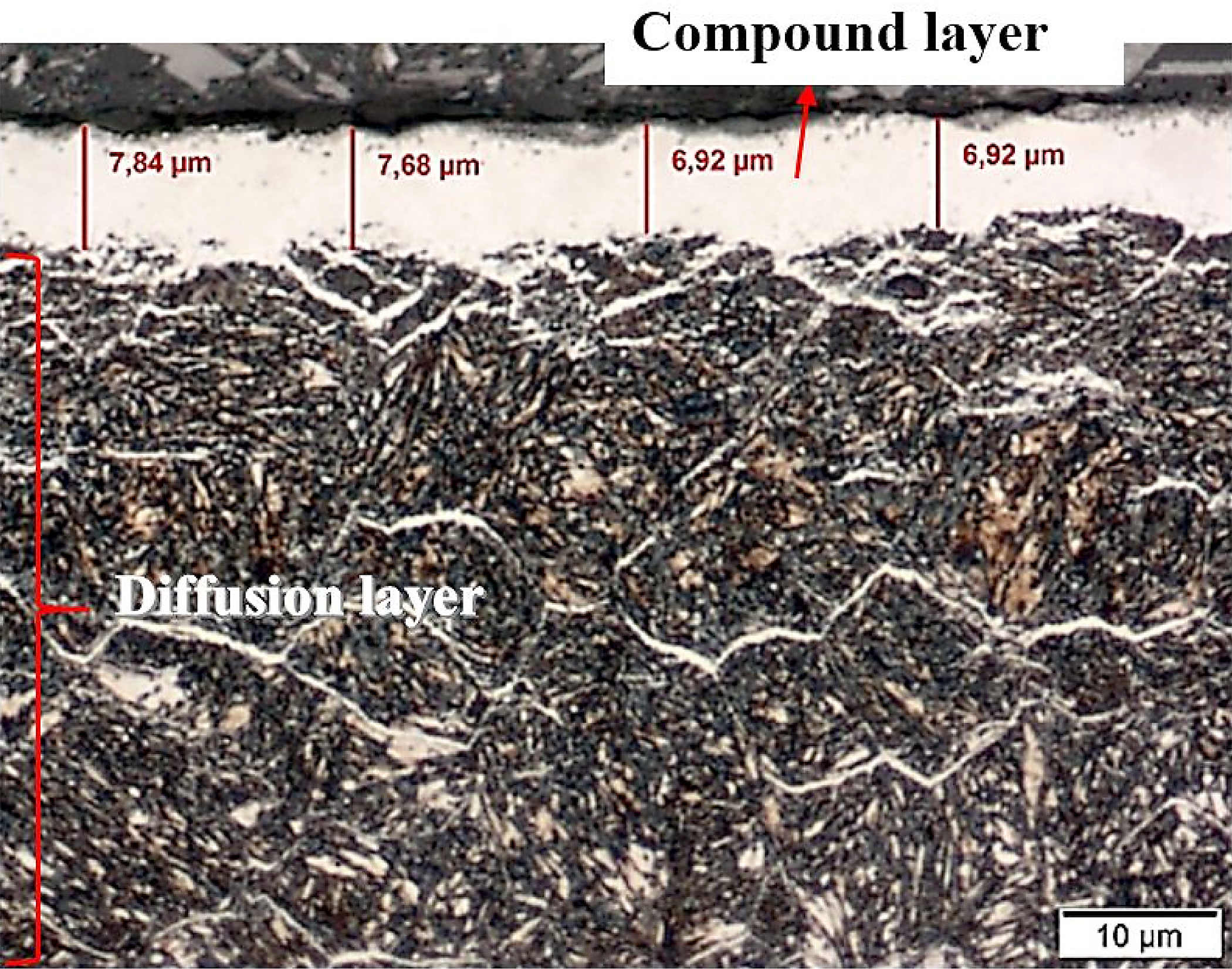
To estimate the nitrogen content in the studied layers, the EDX method of chemical composition analysis with a scanning microscope was applied. The locations where the chemical composition was determined are shown in Figure 2, and quantitative results of chemical composition tests are shown in Table 1. The high nitrogen level and structural phase contrast identified a white (compound) layer.
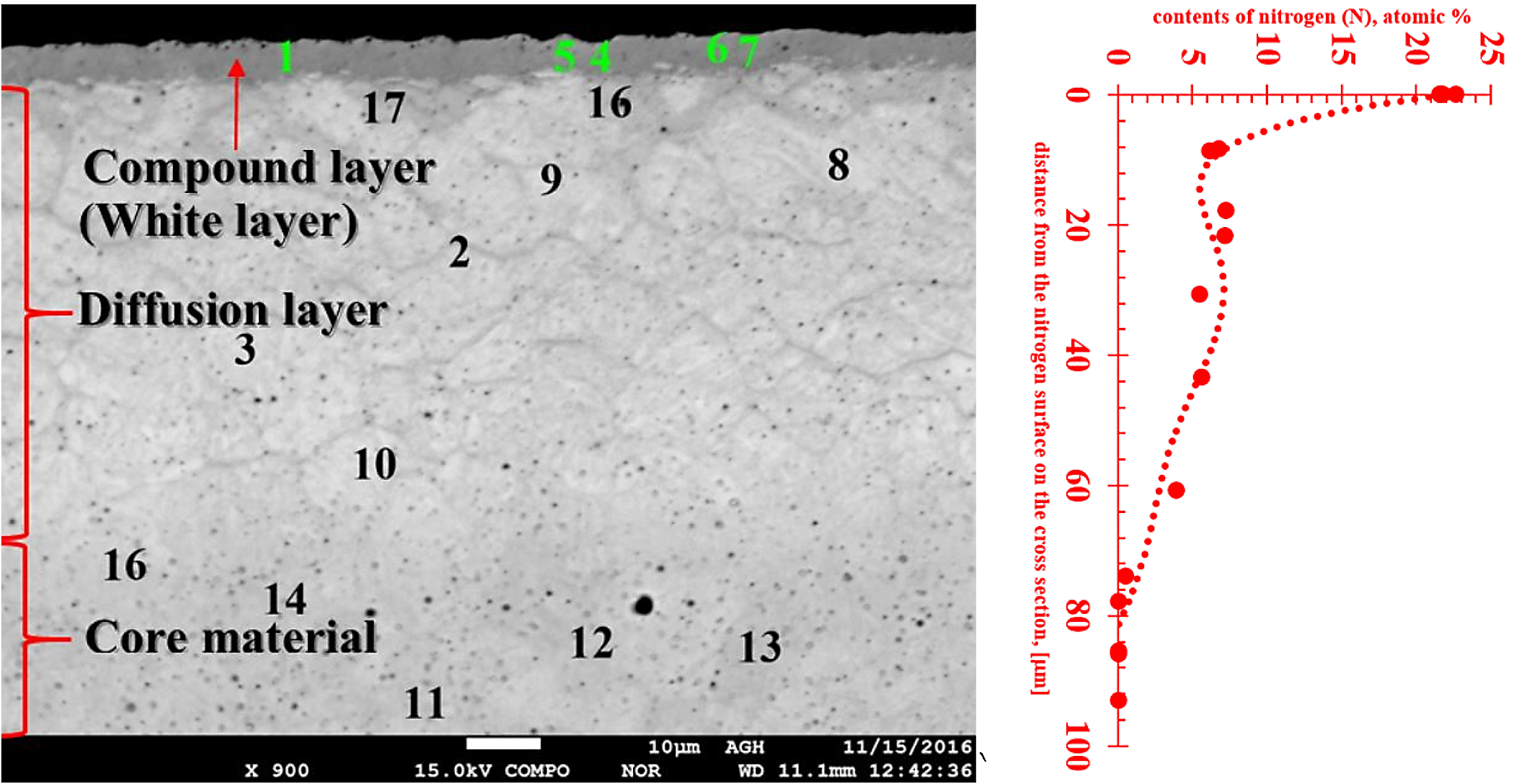
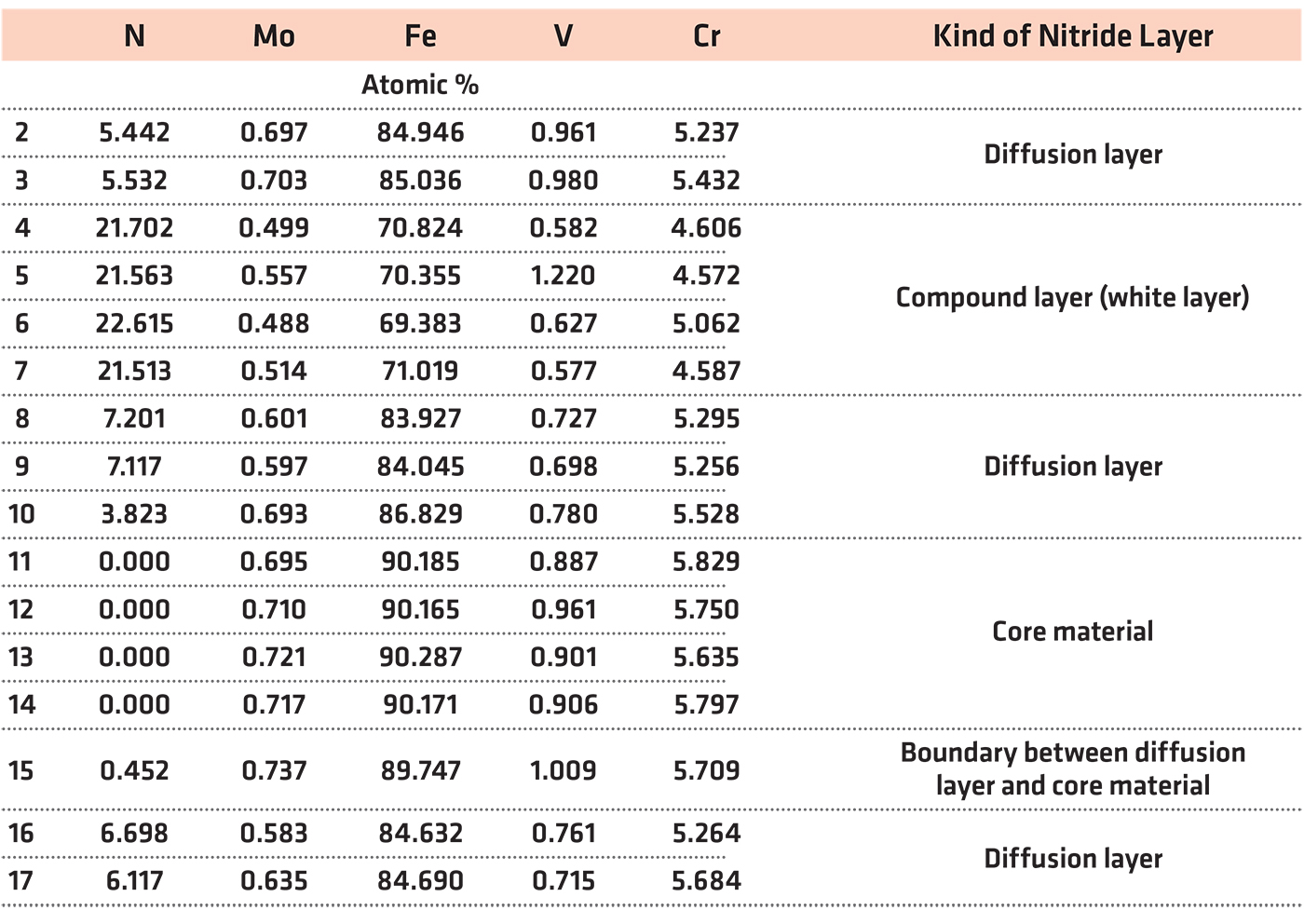
The white layer contained more than 21 at%. of nitrogen, which means the range of occurrence of γ′+ε, or ε-Fe2N nitride, γ′-Fe4N nitride and nitrogen ferrite, which contains 0.01% nitrogen at room temperature (Figure 3). Table 1 also shows results indicating the presence of a diffusion layer with nitrogen in the range 3.9-7.2 at%. These values indicate the extent of the presence of eutectoid mixture α+γ′, consisting of phases: γ′-Fe4N nitride and α-nitride ferrite.
The nitrogen profile attached to Figure 2 clearly shows the very high nitrogen content in the near-surface layer decreases rapidly and already at a distance of about 10 µm from the sample surface, then at a distance of about 80 µm, it reaches the zero level. This is the indicator of the boundary of the diffusion layer. Therefore, the thickness of the hard, nitride layer is relatively small, and the friction forces cause its rapid wear, which makes it necessary to regenerate the layer after a specified period of using the tool.
The results of WDS analysis of the chemical composition of the white layer (compound layer) in the form of elemental distribution maps are presented in Figure 3. In the maps, the position of nitrogen concentration appeared at the same place as the position of Fe, Cr, V, and Mo. The average nitrogen content in the white layer was about 21.84 at% and in the diffusion layer it was about 5.82 at%. The average in both layers’ content of nitrogen was found to be 13.82 at%. The Cr content was estimated in white layer at about 4.71 at%, in the diffusion layer at about 5.35 at%, and in the core material at about 5.75 at% — for an average value of about 5.27 at%. The content of Mo in the white layer was about 0.51 at%, in the diffusion layer about 0.66 at%, and in the core material 0.71 at%. Generally, the content of Mo was 0.63 at%. The V content in the white layer was about 0.75 at% in the diffusion layer 2.03 at% and in the core material about 1.14 at%. The average V content was about 1.31 at%.
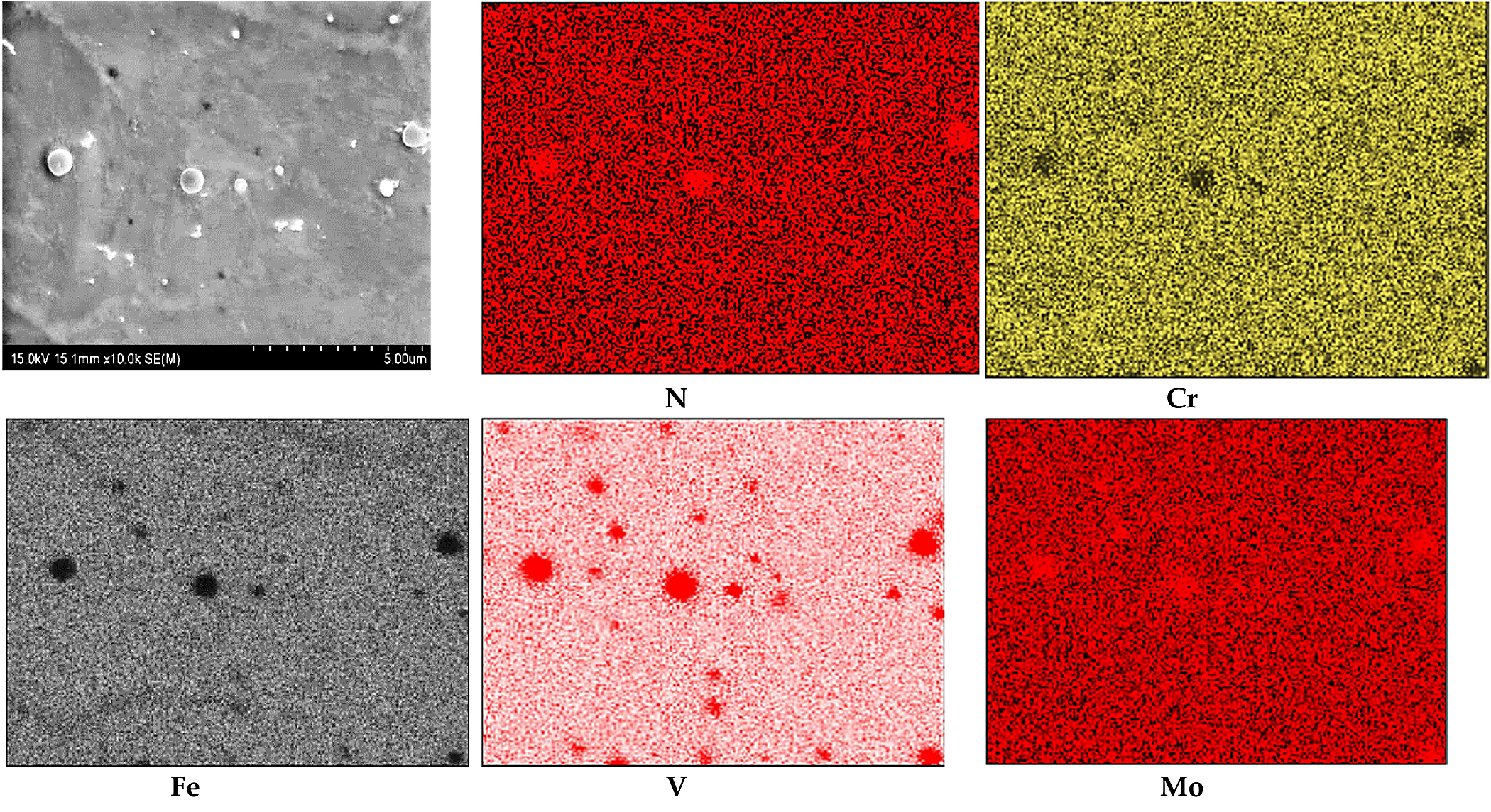
The existence of Cr, V, and Mo in the same positions on the chemical composition maps from WDX measurement (Figure 3) suggests the probability of complex nitride precipitation. In such a case, chromium surely is dissolved in iron nitrides. It was shown that in ternary alloy systems where the crystal structures of the binary boundary nitrides are similar and the interaction parameter difference of the nitride forming elements is moderate, mixed ternary nitrides can develop [27]. Increasing numbers of ternary nitrides, AxMyNz, have been described in recent years, and these exhibit a great richness of structure and physical property [28,29].
After nitriding, the samples were annealed at 470°C for 3 hours (Figure 4a,b), 8 hours (Figure 5a,b), 30 hours (Figure 6a,b), and 60 hours (Figure 7a,b). As a result of annealing, the white layer was degraded. Microstructure observations show the thickness of the white layer decreased as the annealing time increased, and for the annealing time of 30 hours and 60 hours, it disappeared altogether.
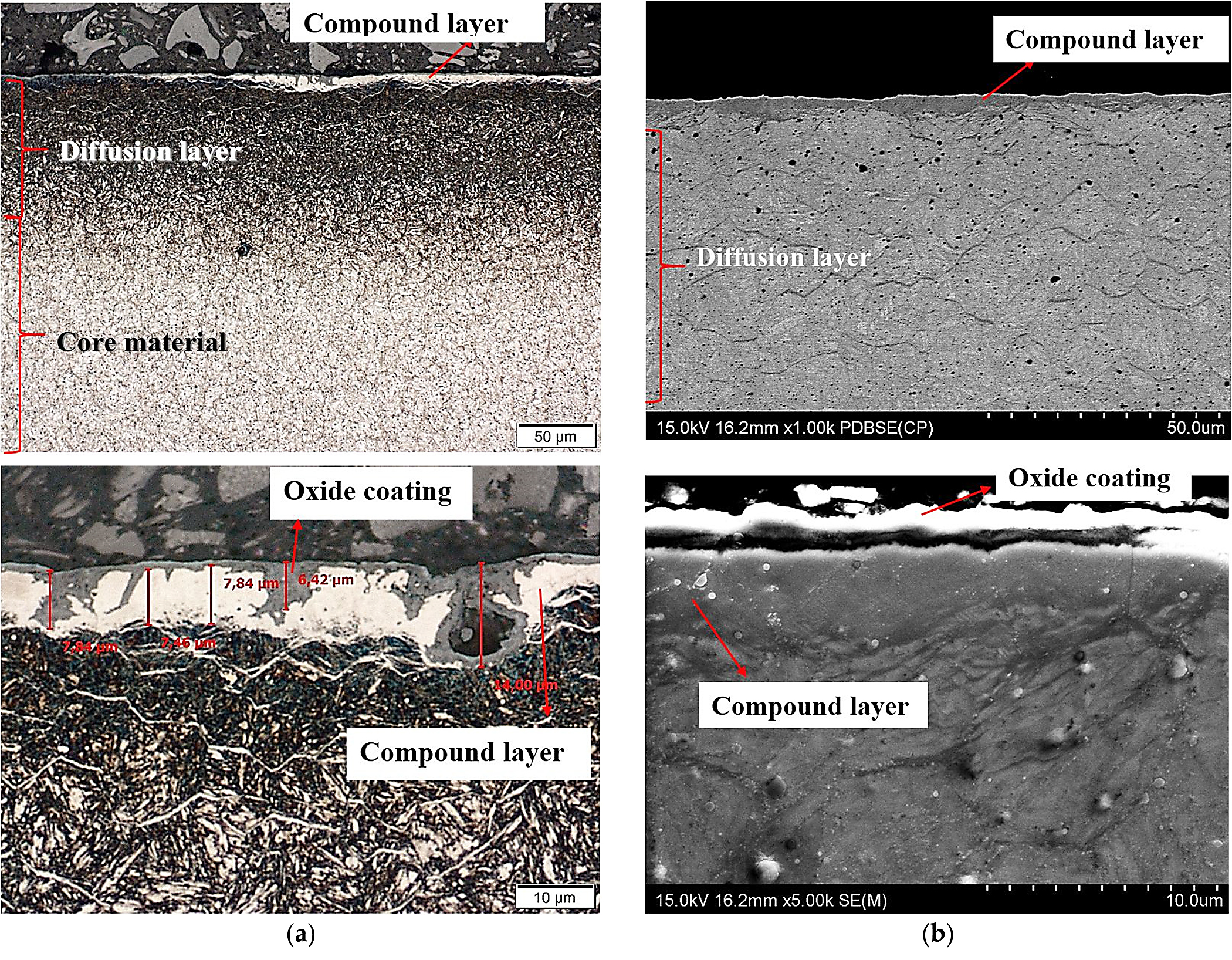
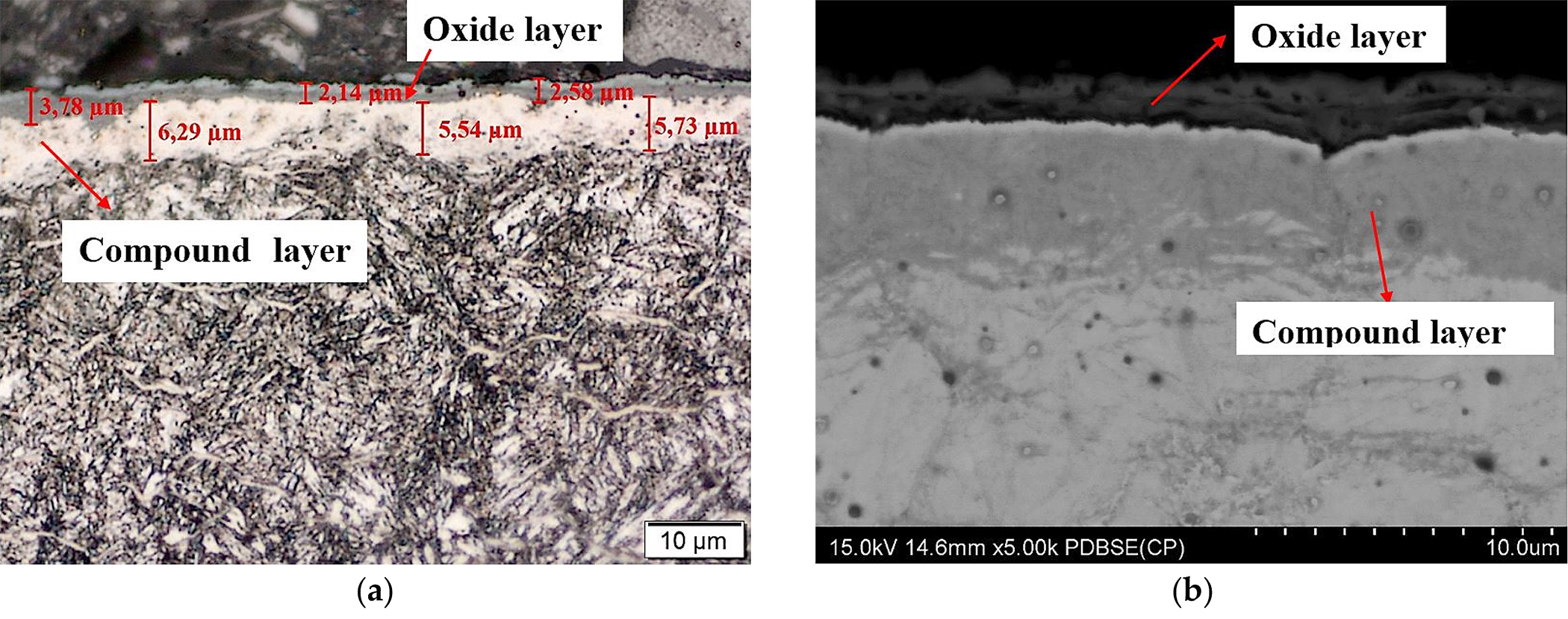
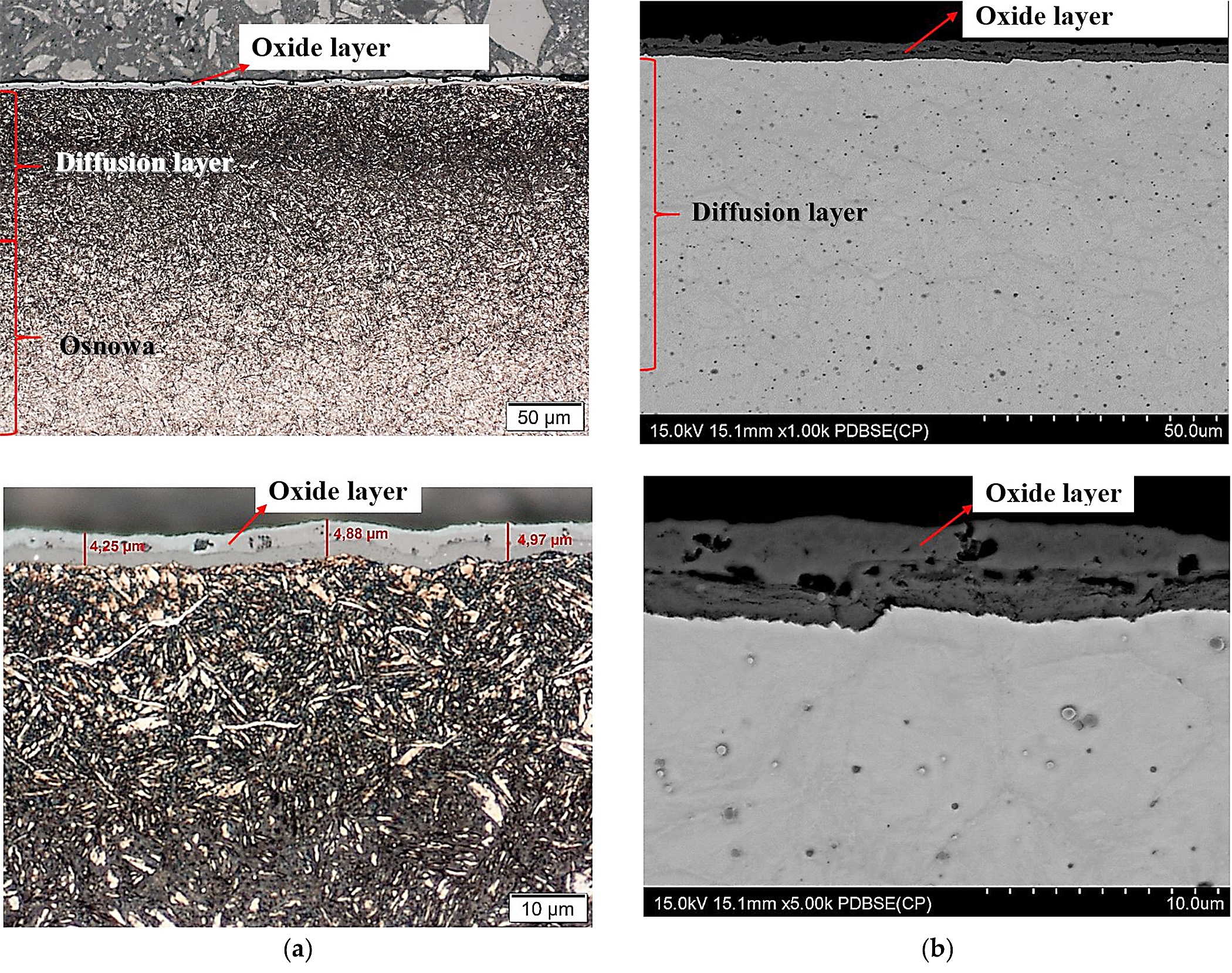
The width of the diffusion layer was observed to grow and equaled about 70 µm for 3 hours and 8 hours of annealing, about 80 µm for 30 hours, and about 100 µm for 60 hours.
The oxidation of nitride samples appeared locally after annealing for 3 hours at 470°C (Figure 4a) and increased with annealing time prolongation. The oxide growth at temperatures between 423 K and 623 K could be described consistently with the oxidation model of Fromhold and Cook (FC) [30]. It describes the initial oxidation of metals (0-20 nm). In the FC theory, the reaction between metal and oxygen takes place at the growing oxide/oxygen interface, requiring both metal ions and electrons to move through the oxide layer to the surface. The transport of electrons can proceed by two mechanisms: tunneling and thermionic emission. For temperatures below 420 K, there is virtually no thermionic emission at the metal-oxide interface, and electron tunneling is the dominant process. According to the FC formalism, at T ≤ 420 K, electron transport proceeds by tunneling through the oxide layer and therefore the limiting film thickness should be nearly independent of the oxidation temperature. However, the measurements show the saturation thickness does depend on the temperature and varies between 8.5 × 1015 O atoms/cm2 at room temperature (RT) and 15 × 1015 O atoms/cm2 at 395 K [31,32]. A two-layer system is formed, with a first layer containing Fe2+ only, and a top layer containing both Fe3+ and Fe2+. The growth of the second layer starts at an oxygen coverage of 4.0 × 1015 O atoms/cm2 and consists of Fe0.77O (which is probably a mixture of FeO and γ-Fe2O3). At higher oxidation temperatures, the relative fraction of Fe3+ in the formed oxide decreases. The oxide layer formed in O2 at 473 K consists of Fe2+ only. The decrease of the oxidation rate coincides with the formation of an oxide layer containing Fe3+. Upon annealing, Fe3+ is reduced to Fe2+, while the displaced Fe0 is oxidized to Fe2+. For larger oxide thicknesses (L > 3 nm) and higher temperatures (T > 420 K), the dominant electron transport processes thermionic emission. For higher temperatures and larger oxide thicknesses, the thermionic emission of electrons is rate-limiting. For lower temperatures and smaller oxide thicknesses, the presence of Fe3+ drastically decreases the oxidation rate. The reduction rate of Fe3+ to Fe2+ increases with increasing temperature. An important feature of the FC theory is the concept of coupled currents: The net electrical current across the oxide layer is zero. At sufficiently high-oxygen pressures, transport of either electrons or metal ions is rate-limiting.
The oxidation of nitride steel was investigated by Yang Li and all [33]. The iron oxide phases of hematite (Fe2O3) and magnetite (Fe3O4) through the post-oxidizing treatment at 400°C and 450°C was found. The hematite has been also identified in work [34] after the post-oxidation of plasma nitriding AISI 4140 steel. In contrast, in [35], after oxidation of nitride layer at 480°C to 500°C, Fe3O4 (magnetite) was reported. It was also described that the precipitation of CrN or Cr in complex nitride precipitations at 500°C removed Cr from the solid solution and adversely affected the oxidation performance at this temperature [35]. Formation of CrN and γ′-Fe4N causes the corrosion resistance of the nitrided layer to decrease [36]. The nitrogen atoms occupy similar types of surface sites as the oxygen atoms [37] Displacement of N atoms from the surface into the bulk by interaction with gaseous O2 will be energetically rather favorable. The bulk diffusion coefficient of N in pure Fe has, at 900 K, a value of about 10-7 cm2/s and an activation energy of 18.9 kcal/mole. Extrapolation to room temperature yields a mean displacement by diffusion of about 5 A within 30 minutes. This process will, however, certainly be strongly accelerated by the rearrangement of the Fe atoms in the course of oxide formation (oxygen chemisorption on Fe(100)), which was found to cause an expansion of the topmost layers, as well as by the energy release associated with the oxygen attack, so that displacement of the N atoms, even at room temperature, becomes feasible. On the other hand, the further growth of the oxide will certainly be retarded by previous formation of a nitride layer in the surface region — this is the well-known anticorrosive effect.
The research showed that, as a result of long annealing times, the surface hardness of the samples decreased by about 200-250 units (Figure 8) in relation to the hardness of the original nitrided sample. The hardness results shown in Figure 8 can be divided into three groups, as presented at Figure 9. The highest hardness (average 1,176 HV) is found in samples annealed for 3, 8, and 12 hours at the temperature of 470°C. The group of samples annealed for 20 hours at 270°C have the average hardness 1,103 HV. The lowest hardness was found in samples annealed for 30 and 60 hours at 470°C with the average hardness 964 HV. The difference between the highest and lowest hardness is 212 HV.
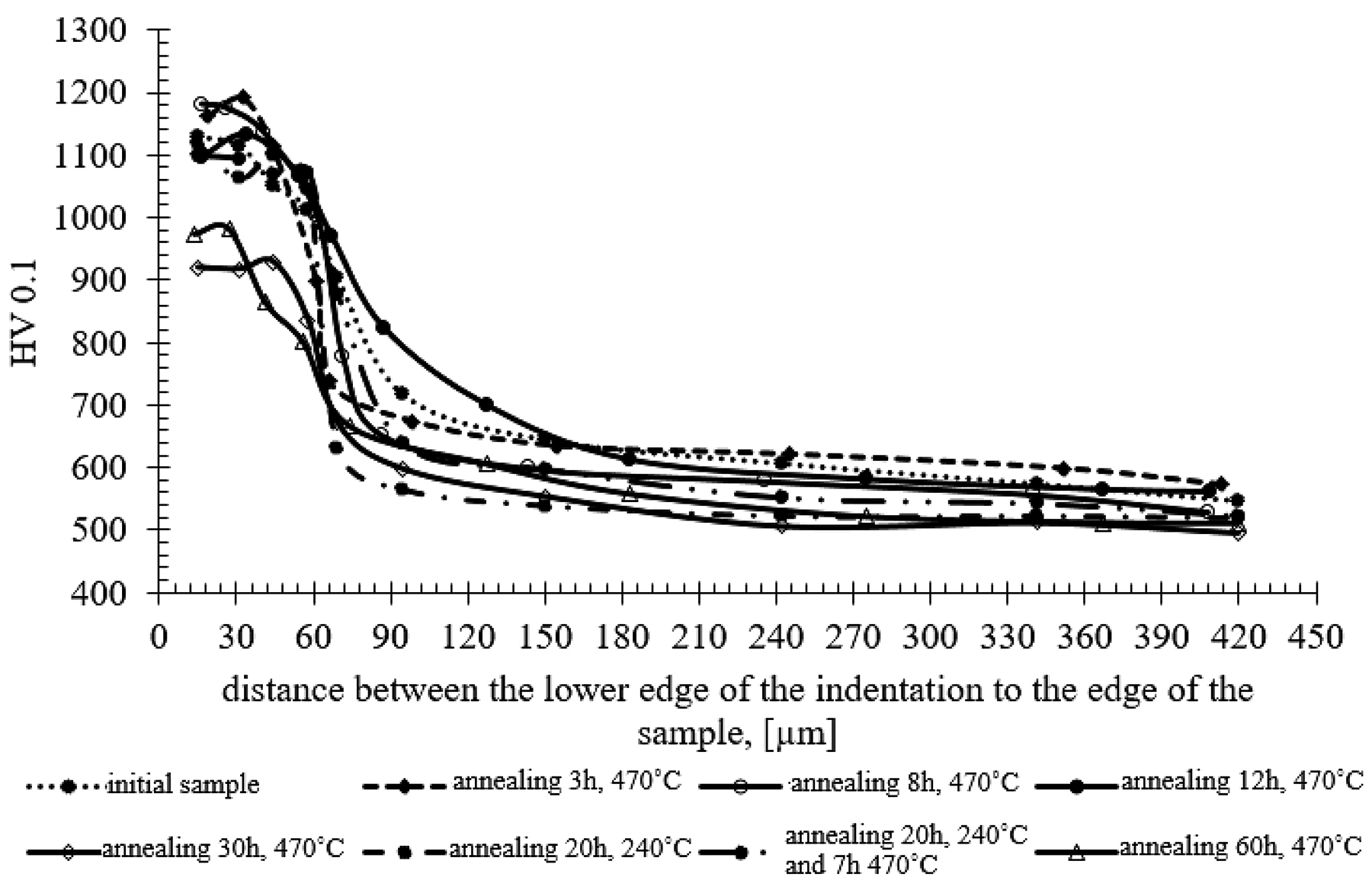
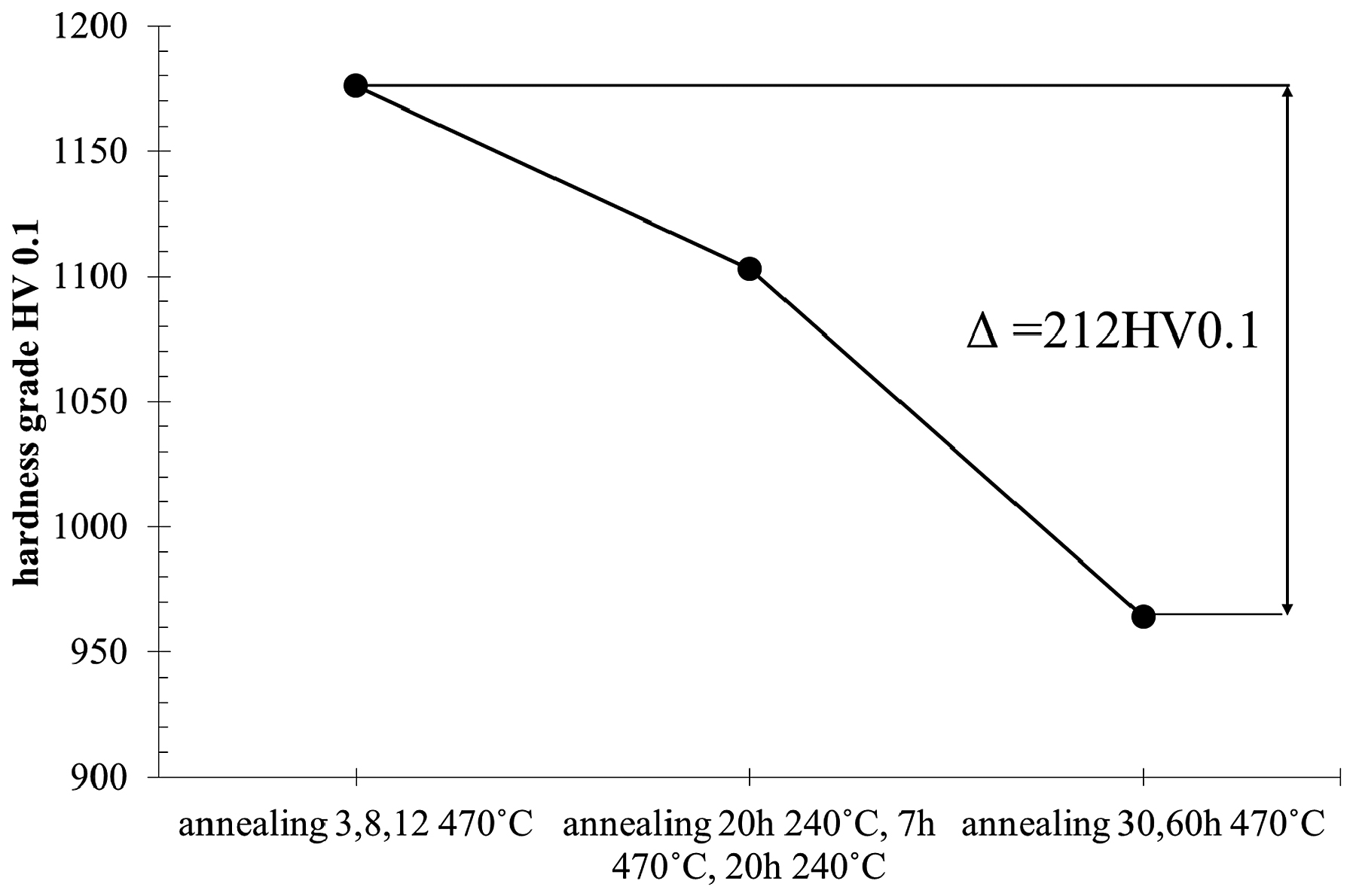
Abrasion tests revealed a correlation between the hardness level and weight loss in the abrasion test. It was found that the lower the hardness, the more abrasive the surface of the samples was (Figure 10).
The analysis of nitrogen content in the white and diffusion layers was based on the results of EDX and WDS chemical composition tests. Figure 11 summarizes the data on nitride content in white and diffusion layers. The white layer contained on average about 19.4% atomic of nitrogen, and this level of nitrogen is stable in samples annealed in the range of 3 to about 12 hours at 470°C. Above this time of annealing, the white layer disappeared. For the annealing times of 30 hours and above, no white layer was found. The nitrogen content in the white layer suggests the occurrence of γ′ + ε phases. When the content of nitrogen decreases, the aforementioned phases do not exist. It should be assumed the disappearance of the white layer occurred as a result of the decrease in nitrogen content, which diffused deep into the substrate, as evidenced by the increase in the thickness of the diffusion layer.
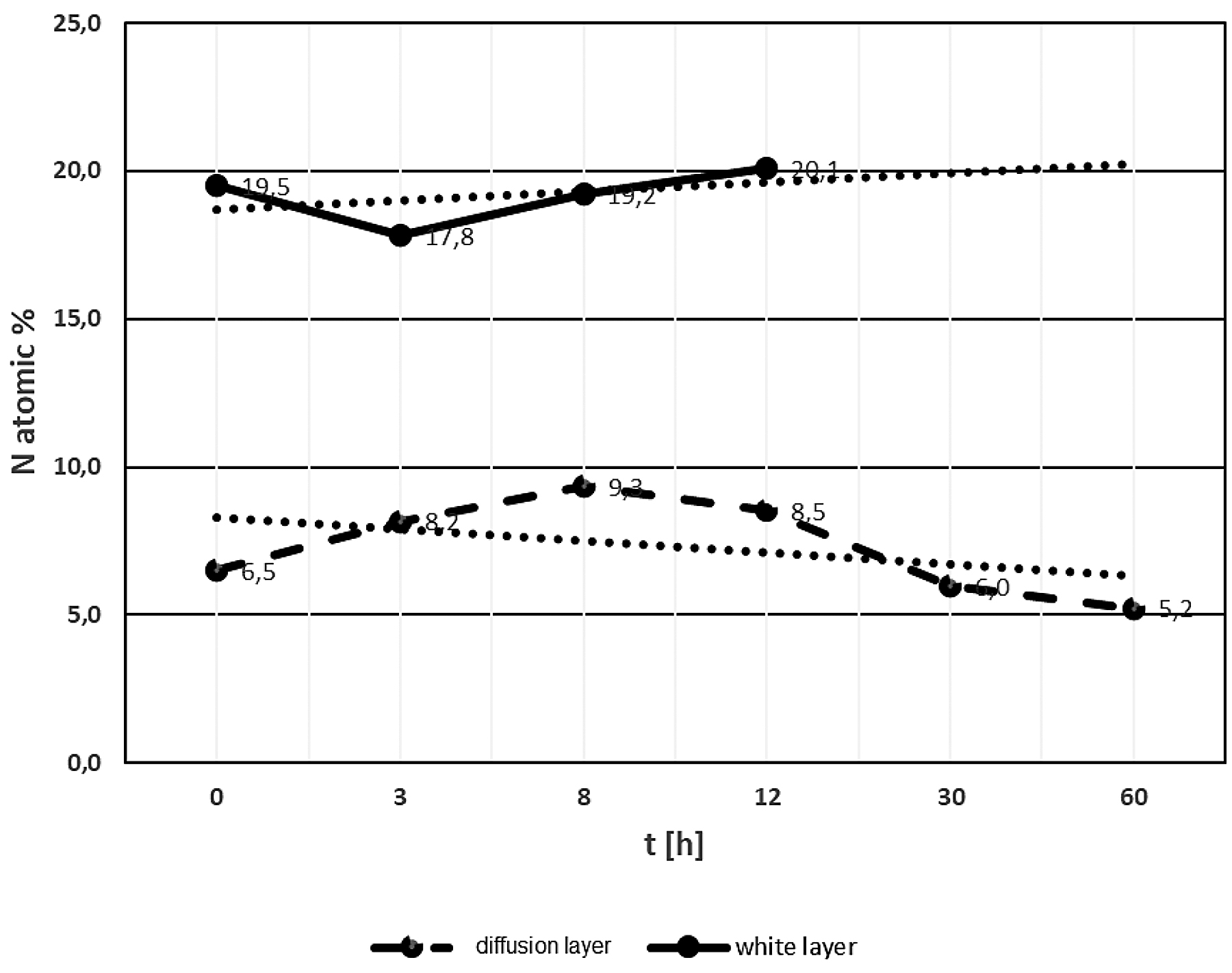
The diffusion layer contained about 8.1% atomic of nitrogen in samples annealed for 8–12 hours (Figure 11). Samples annealed for more than 12 hours showed an average nitrogen content reduced to about 5.6% atomic. Reduced nitrogen content in the diffusion layer predicted the presence of phases α + γ′.
4 Discussion
Coatings and layers produced by various surface engineering methods reveal a variety of structures and thicknesses. This is due to the mechanisms of forming coatings and layers. Nitriding is a specific surface treatment in which the top layer of a tempered tool is saturated with nitrogen at high temperatures. The effect of nitrogen in the atomic structure of steel is the formation of nitrides and the interstitial location of nitrogen in the crystal lattice. Depending on the concentration of nitrogen, different stoichiometric nitride compositions can be found. Apart from nitrides formed as a result of the compound of nitrogen and iron, i.e., nitrides such as Fe2N and Fe4N, moderate mixed ternary nitrides can also develop. In the conducted studies, the searching of nitride particles were performed by EDX and WDS measurement of chemical composition in points and chemical composition maps. We found Cr, Mo, Fe, V, and N concentration in the same places, which suggests formation in or quaternary phase [38,39]. The investigation in work shows that at lower Cr/Mo ratio, hexagonal CrMoN2 precipitation occurred.
The presence in the nitride test layer of a hard nitrogen phases occur near the surface of the samples is significant for die resistance to abrasion. Nitrogen phases especially increase the hardness, which translates into higher abrasion resistance [40]. Reducing the surface nitrogen content in the white layer leads to phase changes and loss of high hardness [41,42]. As a result of experimental annealing, the initial hardness of the nitride layer, amounting to approximately 1,190 HV, was reduced by approximately 210 units (Figure 8). From a practical point of view, reducing the hardness below 1,000 HV disqualifies aluminum extrusion dies for further use. During the annealing at 470◦C, the oxidation of the sample surface develops. The thickness of the oxide layer increases with annealing time prolongation. However, above 8 hours of annealing, it becomes almost stable. Experimental investigations show a “parabolic law” of oxide layer growth [43]. Dwivedi D., Lepková K. and Becker T. [44] point out the importance of texture in the corrosion process. Robert E. Melchers [45] presented different models of corrosion loss starting from different assumptions and showed parabolic and linear lows of oxidation. The oxidation zone containing (Mn,Fe)O and (Mn,Cr,Fe)3O4 oxides in annealed Fe–1.9Mn–1.6Cr steel was investigated by Mao, W., Ma, Y. and Sloof, W.G [46]. It was found the penetration depth of oxides along grain boundaries is much larger (by a factor of two or more) than that of internal oxides formed inside grains. They also found a parabolic rate law of oxidation. In relation to obtained results, it should be assumed the parabolic oxide layer grows and oxidation stabilizes in the range of 30 hours to 60 hours of annealing.
It should be mentioned that the presence of nitride and carbide phases in tools for which no additional heat treatment is applied is beneficial due to the high surface hardness. However, if the surface of the die is designed to be covered with an additional coating, e.g., PVD — Physical Vapor Deposition, the heat treatment should be carried out in such a way that no white layer is formed. There should only be a diffusion zone characterized by maximum effective thicknesses and devoid of carbide precipitations at the grain boundaries of the former austenite [42]. It can therefore be concluded that the design and implementation of the nitriding process and its effects will depend on the purpose for which the tools are used.
The life cycle of the dies includes periods at elevated temperatures (470°C and 520°C) and periods at room temperature. Cyclic changes in the working temperature during die life of the die make it subject to processes of structural and phase changes. This results in changes of properties and, ultimately, after the loss of the required hardness level, the need for regeneration through secondary nitriding.
The substrate is also subject to changes under the influence of temperature. There are recovery processes that reduce dislocation densities and other lattice defects. The processes of precipitation coagulation take place. Precipitations that were coherently bound to the matrix lose their coherence, which results in a decrease of hardness. The phenomena of structure restoration, combined with changes in the morphology of the precipitations, lead to unfavorable changes of properties in terms of die exploitation.
5 Conclusions
On the basis of the research carried out, it was found that:
1. Annealing of the nitrided samples leads to degradation of the nitride layer and the decrease of hardness.
2. Annealing of the samples at 470°C for more than 12 hours causes a decrease in mean hardness value from 1,176 HV to 1,103 HV, and annealing the samples more than 30 hours at this temperature leads to a decrease in hardness to 964 HV.
3. Tests have shown a decrease in abrasive resistance as the annealing time increases.
4. Annealing more than 30 hours at 470°C caused the white layer to disappear and the average nitrogen content in the diffusion layer to decrease to the level of about 6 at%.
Author contributions
Conceptualization, R.H., M.R. and M.W.; Investigations R.H., M.R. and M.W., Writing-Original Draft: M.R., Preparation, R.H., M.R. and M.W. All authors have read and agreed to the published version of the manuscript.
Funding
This research received no external funding.
Acknowledgments
This work was supported by the subsidies for the maintenance and development of research potential of AGH University.
Conflicts of interest
The authors declare no conflict of interest.
References
- Yang, M. Nitriding–Fundamentals, Modeling and Process Optimization. Ph.D. Thesis, Worcester Polytechnic Institute, Worcester, MA, USA, April 2012.
- Conceição Rocha Lima Cesconettoa, M.; Ribeiro Franco, A., Jr.; Aparecido Vieiraa, E. Improving the Abrasive Wear Resistance of a Microalloyed Steel by Plasma Nitriding. Mater. Res. 2015, 18, 334–340. [CrossRef]
- Aydin, H.; Bayram, A.; Topcu, S. The effect of duplex surface treatment on erosion performance of QRO 90 Supreme steel. Mater. Sci. 2013, 19, 19–24.
- Xu, X.; Wang, L.; Yu, Z.; Qiang, J.; Hei, Z. Study of microstructure of low-temperature plasma nitride AISI 304 stainless steel. Metall. Mater. Trans. A 2000, 21A, 1193–1199. [CrossRef]
- Kooi Bart, J.; Somers Marcel, A.J.; Mittemeijer Eric, J. An Evaluation of the Fe-N Phase Diagram Considering Long-Range Order of N Atoms in γ′Fe4N1-x and ε-Fe2N1-z. Metall. Mater. Trans. A 1996, 27A, 1063–1071. [CrossRef]
- Eom, J.Y.; Shankar Rao, V.; Kwon, H.S.; Nam, K.S. Experiments and modeling study on growth behavior of Cr-nitrides formed on electroplated hard Cr during ion-nitriding. J. Mater. Res. 2003, 18, 861–867.
- Widenmeyer, M.; Hansen, T.C.; Meissner, E.; Niewa, R. Formation and Decomposition of Iron Nitrides Observed by in situ Powder Neutron Diffraction and Thermal Analysis. J. Inorg. Gen. Chem. 2014, 640, 1265–1274. [CrossRef]
- Bjørsom, H.B. Investigation of the Compound Layer and Diffusion Zone of Pulsed Plasma Nitrided 51CrV4-Steel. Master’s Thesis, Energy and Nanotechnology Department of Physics, University of Oslo, Oslo, Sweden, September 2013. Available online: https://www.duo.uio.no/bitstream/handle/10852/41371/Bjorsom_ Masteroppgave.pdf?sequence=1 (accessed on 30 September 2013).
- Díaz-Guillén, J.C.; Vargas-Gutiérrez, G.; Granda-Gutiérrez, E.E.; Zamarripa-Piña, J.S.; Pérez-Aguilar, S.I.; Candelas-Ramírez, J.; Álvarez-Contreras, L. Surface Properties of Fe4N Compounds Layer on AISI 4340 Steel Modified by Pulsed Plasma Nitriding. J. Mater. Sci. Technol. 2013, 29, 287–290. [CrossRef]
- Van Hove, R.P.; Sierevelt, I.N.; van Royen, B.J.; Nolte, P.A. Titanium-Nitride Coating of Orthopaedic Implants: A Review of the Literature. J. List Biomed. Res. Int. 2015, 2015, 485975. [CrossRef]
- Caldwell, J.D.; Aharonovich, I.; Cassabois, G.; Edgar, J.H.; Gil, B.; Basov, D.N. Photonics with hexagonal boron nitride. Nat. Rev. Mater. 2019, 4, 552–567. [CrossRef]
- Oliver, E.A. Critical Assessment 23: Gallium nitride-based visible light-emitting diodes. Mater. Sci. Technol. 2016, 32, 737–745. [CrossRef]
- Boukamp, B.A.; Huggins, R.A. Lithium ion conductivity in lithium nitride. Phys. Lett. 1976, 58, 231–233. [CrossRef]
- Drewnicka, D. Advanced investigation methods of nitride layers after heat treatment. Inz˙ynieria Powierzchni (Surf. Eng.) 2012, 2, 14–18.
- Borsa, D.M. Nitride-based Insulating and Magnetic thin Films and Multilayers. Available online: https://www.rug.nl/research/portal/en/publications/nitridebased-insulating-and-magnetic-thin- films-and-multilayers(a667d5ad-8d0b-4eda-80c7-130ef5315665).html (accessed on 10 March 2004).
- Yamashita, S.; Masubuchi, Y.; Nakazawa, Y.; Okayama, T.; Tsuchiya, M.; Kikkawa, S. Crystal structure and magnetic properties of “α″-Fe16N2” containing residual α-Fe prepared by low-temperature ammonia nitridation. J. Solid State Chem. 2012, 194, 76–79. [CrossRef]
- Xiong, X.C.; Redjaïmia, A.; Gouné, M. Transmission electron microscopy investigation of acicular ferrite precipitation in γ′-Fe4N nitride. Mater. Charact. 2010, 61, 1245–1251. [CrossRef]
- Buschow, K.H.J.; Robert, W.; Cahn, P.V. Encyclopedia of Materials: Science and Technology; Elsevier Ltd.: Amsterdam, The Netherlands, 2001.
- Lengauer, W.; Eder, A. Nitrides: Transition Metal Solid-State Chemistry, Encyclopedia of Inorganic Chemistry, 2nd ed.; King, R.B., Ed.; John Wiley & Sons, Ltd.: Chichester, UK, 2005; Volume VI, pp. 3515–3531.
- Litasov, K.D.; Shatskiy, A.; Ponomarev, D.S.; Gavryushkin, P.N. Equations of state of iron nitrides ε-Fe3Nx and γ-Fe4Ny to 30 GPa and 1200 K and implication for nitrogen in the Earth’s core. J. Geophys. Res. Solid Earth 2017, 122, 3574–3584. [CrossRef]
- Jegoua, S.; Barrallier, L.; Kubler, R. Phase transformations and induced volume changes in a nitrided ternary Fe–3%Cr–0.345%C alloy. Acta Mater. 2010, 58, 2666–2676. [CrossRef]
- Runge, J.M.; Pomis, A.J. Presented at the XII Ebrats Brasilian Surface Treatment Meeting and II Latin—American Interfinish, Sao Paulo, Brazil, 10 May 2006.
- Kugler, G.; Vecko–Pirtovsek, T.; Tercelj, M. Wear behavior of nitrided microstructures of aisi h13 dies for hot extrusion of aluminum. Metallurgija 2006, 45, 21–29.
- Liapina, T.; Mittemeijer, E.J.; Leineweber, A.; Knapp, M. γ′,-Fe4N formation in decomposing ε-Fe3N: A powder diffraction study using synchrotron radiation. Z. FÜR Krist. Suppl. 2006, 23, 449–454. [CrossRef]
- Available online: https://www.alliedmarketresearch.com/aluminium-market (accessed on 20 December 2019).
- Available online: https://www.grupakety.com/Media/files/PROGNOZA_3.pdf (accessed on 8 February 2019).
- Steiner, T.; Mittemeijer, E.J. Alloying element nitride development in ferritic Fe-based materials upon nitriding: A review. J. Mater. Eng. Perform. 2016, 25, 2091–2102. [CrossRef]
- Clarke, S.J. Encyclopedia of Materials: Science and Technology; Buschow, J.K.H., Cahn, R.W., Veyssiere, P., Flemings, M.C., Eds.; Elsevier Ltd.: Amsterdam, The Netherlands, 2001.
- Farault, G.; Gautier, R.; Baker, C.F.; Bowman, A.; Gregory, D.H. Crystal Chemistry and Electronic Structure of the Metallic Ternary Nitride, SrTiN2. Chem. Mater. 2003, 15, 3922–3929. [CrossRef]
- Fromhold, A.T., Jr. Fundamental Theory of the Growth of Thick Oxide Films on Metals. J. Phys. Soc. Jpn. 1980, 48, 2022–2030. [CrossRef]
- Roosendal, S.J. Passivation Mechanisms in the Initial Oxidation of Iron by Oxygen and Water Vapor. Ph.D. Thesis, University Utrecht, Utrecht, Netherlands, 1999.
- Chemical kinetics. In Reactions of Solids with Gases; Bamford, C.H.; Tipper, C.F.H.; Compton, R.G. (Eds.) Elsevier: Amsterdam, The Netherlands; Oxford, UK; New York, NY, USA; Tokyo, Japan, 1984; Volume 21.
- Li, Y.; Wang, L.; Zhang, D.; Shen, L. The effect of surface nanocrystallization on plasma nitriding behaviour of AISI 4140 steel. Appl. Surf. Sci. 2010, 256, 4149–4152. [CrossRef]
- Castro Munoz, A.E.; Piedad-Beneitez, A.; Valencia-Alvarado, R.; López-Callejas, R.; Mercado-Cabrera, A.; Pena-Eguiluz, R.; Rodriguez-Mendez, B.G. The Corrosion Enhancement due to Plasma Post-Oxidation Subsequent to Plasma Nitriding of a Steel AISI 4140. Acta Phys. Pol. 2015, 128, 824–827. [CrossRef]
- Cao, Y.; Norell, M. Role of Nitrogen Uptake During the Oxidation of 304L and904L Austenitic Stainless Steels. Oxid. Metals 2013, 80, 479–491. [CrossRef]
- Yuan, X.; Zhao, Y.; Li, X.; Chen, L. Effects of gas nitriding temperature on the surface properties of a high manganese TWIP steel. Metals 2017, 7, 102. [CrossRef]
- Ertl, G.; Huber, M. Interaction of Nitrogen and Oxygen on Iron Surfaces. Z. Fur Phys. Chem. Neue Folge 1980, 119, 97–102. [CrossRef]
- Kniep, R. Ternary and quaternary metal nitrides: A new challenge for solid state chemistry. Pure Appl. Chern. 1997, 69, 185–191. [CrossRef]
- Steiner, T.; Meka, S.R.; Bischoff, E.; Waldenmaier, T.; Mittemeijer, E.J. Nitriding of ternary Fe–Cr–Mo alloys; role of the Cr/Mo-ratio. Surf. Coat. Technol. 2016, 291, 21–33. [CrossRef]
- Tacikowski, J.; Wach, P.; Michalski, J.; Burdyn´ski, K. Separation hardening by heat treatment of nitrided carbon steel. Mater. Eng. 2014, 6, 552–555.
- Andrzejewska, E.; Gonzalez-Arrabal, R.; Borsa, D.; Boerma, D.O. Study of the phases of iron–nitride with a stoichiometry near to FeN. Nucl. Instrum. Methods Phys. Res. Sect. B Beam Interact. Mater. At. 2006, 249, 838–842. [CrossRef]
- Orlik, R.; Ratajski, J.; Suszko, T.; Michalski, J.; Dobrodziej, J.; Gilewicz, A. Precise shaping of phase structure and thickness of nitrided layer on the example of dies for pressure casting of aluminium. Surf. Eng. 2009, 3, 15–20.
- Xu, C.H.; Gao, W. Pilling-Bedworth ratio for oxidation of alloys. Mater. Res. Innov. 2000, 3, 231–235. [CrossRef]
- Dwivedi, D.; Lepková, K.; Becker, T. Carbon steel corrosion: A review of key surface properties and characterization methods. RSC Adv. 2017, 7, 4580–4610. [CrossRef]
- Melchers, R.E. Predicting long-term corrosion of metal alloys in physical infrastructure. NPJ Mater. Degrad. 2019, 3, 1–17. [CrossRef]
- Mao, W.; Ma, Y.; Sloof, W.G. Internal Oxidation of Fe–Mn–Cr Steels, Simulations and Experiments. Oxid. Metals 2018, 90, 237–253. [CrossRef]
Published May 27, 2020. © 2020 by the authors. Licensee MDPI, Basel, Switzerland. This article is an open access article distributed under the terms and conditions of the Creative Commons Attribution (CC BY) license (http://creativecommons.org/licenses/by/4.0/). The article was edited to conform to the style of Thermal Processing magazine. The original article is available at: www.mdpi.com/1996-1944/13/10/2311?type=check_update&version=3